The Transport Characteristics of 238U, 232Th, 226Ra, and 40K in the Production Cycle of Phosphate Rock
Article information
Abstract
Background
Phosphate rock and its by-product are widely used in various industries to produce phosphoric acid, gypsum, gypsum board, and fertilizer. Owing to its high level of natural radioactive nuclides (e.g., 238U and 226Ra), the radiological safety of workers who work with phosphate rock should be systematically managed. In this study, 238U, 232Th, 226Ra, and 40K levels were measured to analyze the transport characteristics of these radionuclides in the production cycle of phosphate rock.
Materials and Methods
Energy dispersive X-ray fluorescence and gamma spectrometry were used to determine the activity of 238U, 232Th, 226Ra, and 40K. To evaluate the extent of secular disequilibrium, the analytical results were compared using statistical methods. Finally, the distribution of radioactivity across different stages of the phosphate rock production cycle was evaluated.
Results and Discussion
The concentration ratios of 226Ra and 238U in phosphate rock were close to 1.0, while those found in gypsum and fertilizer were extremely different, reflecting disequilibrium after the chemical reaction process. The nuclide with the highest activity level in the production cycle of phosphate rock was 40K, and the median 40K activity was 8.972 Bq·g−1 and 1.496 Bq·g−1, respectively. For the 238U series, the activity of 238U and 226Ra was greatest in phosphate rock, and the distribution of activity values clearly showed the transport characteristics of the radionuclides, both for the byproducts of the decay sequences and for their final products.
Conclusion
Although the activity of 40K in k-related fertilizer was relatively high, it made a relatively low contribution to the total radiological effect. However, the activity levels of 226Ra and 238U in phosphate rock were found to be relatively high, near the upper end of the acceptable limits. Therefore, it is necessary to systematically manage the radiological safety of workers engaged in phosphate rock processing.
Introduction
The National Academy of Science (1987) has pointed out that the various naturally occurring radioactive materials (NORM) in the environment are the major origin of annual radiation exposure (e.g., approximately 82% of total radiation) [1]. Although the total radioactivity concerned with NORM might be less than those affected by artificial radionuclides from earlier nuclear weapon testing or accidents of the nuclear power production (NPP) facilities, systematic management to reduce long-term or high dose exposure to workers is strongly required.
In response to these concerns, various policies, regulations, and guidelines regarding NORM have been enacted or proposed worldwide. In particular, based on the Euratom 96 directive, some European countries either enforce regulations or are institutionalizing guidelines for the systematic tracking including the production, management, and distribution of NORM [2–4]. Domestically, since 2012, guidelines of ‘Radioactive Safety Management of Environmental Radiation’ suggested by the Korean Nuclear Safety and Security Commission have been initiated for the systematic management of NORM.1), The guidelines aim to reduce the radiation exposure incurred to both the general public and workers of the raw materials, NORM-containing products, and by-products enriched with the specific radioactive nuclides. Radioactive exposure from the process of manufacturing, handling, and managing NORM-containing products refers to not only external exposure, but also to internal exposure from inhalation and ingestion. Workers involved in NORM manufacturing are certainly exposed to large amounts of radiation during the pulverization or transportation process. Because pulverized radioactive materials with a disproportionately larger surface area can be easily deposited along the respiratory tract, severe health complications occur owing to the internal exposure [5].
The ‘Radioactive Safety Management of Environmental Radiation’ guideline requires that the concentration of 238U, 235U, 232Th, or their radioactive progeny in NORM or by-products should not exceed 1.0 Bq·g−1 (10 Bq·g−1 for 40K). The guideline also limits the aggregate amount of radioactivity to above 1000 kBq (for 238U, 235U, and 232Th) or 10,000 kBq (for 40K). The guideline requires that the annual radiation exposure from the processed NORM-containing products not exceed 1 mSv, individually.
It is well known that a phosphate rock, which has highly enriched radioactivity with raw materials (e.g., bauxite, zircon, and monazite), is buried throughout China, Morocco, South Africa, United America, etc. The phosphate rock distributed in domestic industries is mainly imported from these countries [6].
Although the radioactivity of phosphate rock varies vastly with their geographical origin, the radioactive concentrations of 238U, 232Th, and 226Ra have been reported as the following ranges of 0.1–4.0 Bq·g−1, 0.015–0.15 Bq·g−1, and 0.6–3.0 Bq·g−1, respectively. In some cases, the radioactivity in phosphate rock distributes an extremely high level [7]. Consequently, the International Atomic Energy Agency (IAEA), the International Commission on Radiological Protection (ICRP), and the European Union have proposed safety guidelines on the NORM industry including phosphate rock [2, 8]. According to the previous report, nevertheless, the annual radiation exposure dose might be different with the specific process, and the highest exposure dose associated with phosphate rock has been found in storage containers (a maximum value of 0.8 mSv), followed by transportation cargo (0.05 mSv), phosphoric acid production rooms (0.02 mSv), and gypsum-drying rooms (0.05 mSv) [9].
It is well-known that phosphate rock as a sedimentary rock biochemically originates with the major components of P2O5 and CaO. Phosphate rock is generally used as raw materials for fertilizers as well as in construction materials, phosphoric acid production, medicine, semiconductors, ceramics, and silk production [10].
Figure 1 illustrates the interesting radionuclides across the various stages of the production cycle of phosphate rock. The physicochemical processing of phosphate rock makes two types of by-products: phosphoric acid and phospho-gypsum. According to the Ministry of the Environment in KOREA, the radon dose in boards has been detected at 25-times greater than in gypsum boards as building construction materials containing phospho-gypsum [11]. The dose of 40K radiation in compound fertilizer from phosphoric acid is also high owing to the KCl addition processes [1].
In general, gamma spectrometry, a liquid scintillation counter (LSC), alpha spectrometry, inductively coupled plasma mass spectrometry (ICP-MS) and X-ray fluorescence spectrometry (ED-XRF) were used to evaluate the radioactivity of 238U, 232Th, 226Ra, and 40K. In particular, nevertheless, the alpha spectrometry, LSC, and ICP-MS are primarily useful for analyzing the low level of radioactivity, and they require complex and time-consuming sample pretreatment such as acid digestion, purification, separation, and dilution. In contrast, as analytical non-destructive methods, gamma spectrometry and ED-XRF permit the determination of radionuclides without complicated and time-consuming radiochemical separations [12]. As the point of the analytical characteristics, the determination method of 238U, 232Th, and 226Ra can be divided into direct or indirect methods. While a direct method evaluates the radioactivity based on the determined results for the specific target nuclide, an indirect analysis, assuming secular equilibrium, calculates the radioactivity based on those of its daughter nuclides. However, an indirect measurement might cause inaccuracies owing to the radioactive disequilibrium in a spectrometry measurement [13, 14].
In this study, to lay the groundwork for future studies assessing the radiological safety of natural radionuclides, we investigated the radioactivity distribution characters of 238U, 232Th, 226Ra, and 40K in the production cycle of phosphate rock (e.g., raw materials, phospho-gypsum, gypsum board, and fertilizer). The radioactive disequilibrium that may occur among nuclides in the production cycle in an indirect spectrometry evaluation may limit the applicability of our findings. Thus, to assess the effect of radioactive disequilibrium on the reliability of our data, direct measurements using ED-XRF and indirect measurements using gamma spectrometry were simultaneously determined and compared.
Materials and Methods
1. Sample pretreatment
To evaluate the status of the radioactivity in NORMs at various points of the production cycle, the following samples reflecting different stages of the cycle were analyzed: 1) 23 types of raw phosphate rock; 2) 24 types of gypsum in phosphoric acid manufacturing; 3) 19 types of k-related fertilizer; 4) 7 types of fertilizer; and 5) 13 types of gypsum board currently distributed in Korea. Samples were homogenized with a milling machine (8530 Enclosed Shatterbox, SPEX SampePrep, Metuchen, NJ) and dried for over 3 hours at 105°C. The samples were separately prepared for gamma spectrometry and ED-XRF. While the samples for gamma spectrometry were filled in aluminum containers with about 100 g and the mount sealed, the samples for ED-XRF were filled in plastic cylindrical sample cups (ф of 32 mm; height of 1 cm) encased with a Mylar film.
2. Gamma spectrometry of NORM
Although the alpha decay of the parent nuclides 238U (T1/2= 4.468×109 y) and 232Th (T1/2=1.402×1010 y) in NORMs is associated with transitions in the energy levels of their radioactive progeny, the actual gamma emission is very small owing to the high rates of the internal conversion; this makes it unfeasible to use standard high-purity germanium (HPGe) detectors to perform direct measurements [15]. Although the alpha decay of 226Ra (T1/2=1.6×103 y) into 222Rn (T1/2=3.8 d) could be apparently detected at 186.2 keV, 235U (T1/2=7.04×108 y) of the 185.7 keV gamma peak may interfere in an ordinary case [16]. Therefore, to accurately quantify the gamma peaks of 226Ra, it is necessary to perform a complex calculation to adjust the ROIs for the interference peaks. However, 235U and 40K, which emit the prominent gamma emissions, could be easily determined in the gamma spectrometry. For the 238U, 226Ra, and 232Th nuclides, secular equilibriums of the radioactivity of specific radioactive progeny within the decay series were used [17, 18].
Typically, the phosphate rock was treated in the process reflecting the following reaction: Ca3(PO4)2 reacts with phosphoric acid to produce CaH4(PO4)2 (Equation 1), and CaH4 (PO4)2 reacts with sulfuric acid to produce phosphoric acid and gypsum (Equation 2).
As mentioned above, an imbalance in secular equilibrium might occur in raw materials, by-products, or NORM-containing products as a result of various geochemical or physicochemical treatments. After as long as 7-times the half-life of the progeny, the secular equilibrium between all of the nuclides in the decay series was reached. For instance, the growing back of secular disequilibrium induced by the decay of 238U to 234Th (T1/2=27 d) takes around 6 months. In case of the disequilibrium induced by the decay of 232Th into 228Ra and 228Ac, although the half-life of 228Ac is only 6 min, it would take over 40 years to achieve secular equilibrium owing to the long half-life of 228Ra (T1/2=5.7 years). 222Rn and 220Rn, a radioactive progeny of 226Ra and 224Ra (T1/2=1.6 years), exist as inert gases in the environment and are often found high levels in raw material. Thus, secular disequilibrium almost always occurs. Therefore, to prevent disequilibrium by the leakage of radon gas, the aluminum container filled samples were completely sealed to prevent radon leakage and left for at least more than 3 weeks. This time-consuming pretreatment is the one of the major limitations in gamma spectrometry.
In this study, an N-type HPGe (EG&G ORTEC, Oak Ridge, TN) detector and MCA were used for gamma spectrometry. A calibration standard was prepared with a standard reference solution (Eckert & Ziegler, Berlin, Germany). The energy and efficiency of the detector were calibrated using the calibration standard with the same geometry and chemical composition.
The empty aluminum containers were periodically measured for correcting the background count rate. The minimum detectable activity (MDA) of the system used was calculated with the Equation 3. The evaluated MDA of 226Ra, 228Ac, and 40K were 0.004 Bq·g−1, 0.005 Bq·g−1, and 0.14 Bq·g−1, respectively.
Nb: Background count rate (cps)
T: Activity (Bq)
ɛ: Counting efficiency
Wsmp: Sample weight (g)
3. Analysis of the radioactivity using ED-XRF
The radioactivity of 238U and 232Th in raw materials, by-products, and NORM-containing products were quantitatively analyzed using ED-XRF. The ED-XRF method, which is able to determine metallic elements qualitatively and quantitatively, is representative of the non-destructive methods for multi-elemental analysis simultaneously [19]. It is possible to measure all elements from 23Na to 238U without restriction of the sample size. Typically, a sample pretreatment is rarely needed for solid powder. However, the variation of composition and characteristics of the sample medium play a critical role in the analytical results.
The required measurement time for each sample in the ED-XRF methods is approximately 600 seconds for mass scanning and calculation of all elements. The concentration of U and Th were calculated with a counting rate of a specific Lα-X-ray at 13.612 keV and at 12.967 keV, respectively. Because the peak overlap occurred at 13.612 keV owing to the high content of 85Rb and 208Pb, it should be separated by removing the characteristic potential peak from the U peak using their specific Kα-X-ray. The measurement and calculation were automatically performed using the X-LapPro program. The calibration curves for U and Th in 105 dynamic ranges were created using various standard references materials of soil and rock media. The resulted determination coefficient (r2) of calibration curves for them were 0.9981 and 0.9946, respectively. The detection limit was around 1 mg·kg-1 (≅238U, 0.012 Bq·g−1 and 232Th, 0.041 Bq·g−1).
The calculation of the mass-radioactivity relationship (specific activity), which uses the decay time of the nuclide and the atomic weight, is presented in Equation 4. The specific activity of 238U (a relative isotopic abundance of 99.3% was applied) was 12.446 Bq·g−1, and that of 232Th was 4.007 Bq·g−1.
m: Mass of analyte
A: Activity (Bq)
M: Molar mass (g)
t1/2: Half-life (s)
NA: Avogadro’s number
Results and Discussion
1. Application of analytical methods considering disequilibrium
In general, 80% of 226Ra, 14% of 238U, and 30% of 232Th in phosphate rock tend to be enriched in phospho-gypsum and the rest remain in phosphoric acid through the manufacturing process. In particular, because the solubility product (Ksp) of Ra with sulfate is extremely low (3.66×10−11), it easily precipitates as RaSO4. Thus, large amounts of Ra would be concentrated in phospho-gypsum, while U and Th are transferred into phosphoric acid. Through these chemical reaction processes, the secular equilibrium between specific decay series breaks down [20, 21]. In the case of gypsum boards made by mixing the phospho-gypsum, sawdust and lightweight material, the radioactivity of Ra in the gypsum boards could be higher than that of 238U. On the other hand, for the phosphoric acid used to manufacture compound fertilizers, the radioactivity of 238U could be higher than that of 226Ra.
The standard reference materials (SRM) with soil, phosphate rock, and bauxite media of the National Institute of Standards and Technology (NIST, Gaithersburg, MA) were used for the QA/QC of ED-XRF and gamma spectrometry. For U and Th, the relative errors of both methods for SRMs were below ±20% compared to the certified/referenced values. On the basis of the validation guidelines for the radioactivity analysis [22, 23], the analytical results were reasonably reliable.
In order to evaluate the applicability of ED-XRF and gamma spectrometry, the comparative results of the activity concentrations between both methods in the 238U series (A) and 232Th series (B) are presented in Figure 2. For the purpose of spectral certainty, the comparative results in a low concentration range were expanded in 238U series.
With the indirect measurement method, the radioactivity of 238U could be calculated using 226Ra. If the mother and progeny nuclides were in the secular equilibrium, their radioactivity should be identical. This fact means that if the regression coefficient is around 1, the radioactivity of the progeny nuclides (e.g., 226Ra) could be directly used for the mother nuclides (e.g., 238U) under secular equilibrium. In phosphate rock samples, with relatively high radioactivity, the regression coefficient of 226Ra and 238U (226Ra/238U) is 0.86±0.13. The coefficient of determination is 0.909. This finding indicates that secular equilibrium was achieved between the mother nuclide and radioactive progeny in the phosphate rock (Figure 2A). Thus, the 238U concentrations could be deduced by the 226Ra activity in the gamma-ray spectrometry. In contrast, in gypsum samples, the 226Ra/238U ratio was 5.56±3.93, indicating that the concentrations of 226Ra were about 5-times higher than those of 238U. The 226Ra/238U ratio in fertilizers and gypsum board samples were 0.06±0.05 and 2.24±1.70, respectively. Thus, one may conclude that the secular disequilibrium between 226Ra and 238U in gypsum, fertilizers, and gypsum board is obvious (Figure 2C).
Because 228Ra is also enriched in gypsum and 232Th and 228Ac are dissolved in phosphoric acid, disequilibrium between 232Th and 228Ac could be observed owing to the loss of 228Ra in the process. Because the half-life of 228Ac is only 6.1 min, the radioactivity of 228Ac in gypsum is restored quickly and reached secular equilibrium with 228Ra. However, the half-life of 228Ra is 5.7 years, and it takes longer for secular equilibrium to be restored; thus, 232Th-228Ra disequilibrium occurs. As shown in Figure 2B, the determination coefficient between 232Th and 228Ac was 0.875 indicating a high relationship between both methods. The ratio (228Ac/232Th) was 0.91±0.29 in phosphate rock, 0.79±0.39 in gypsum, and 0.75±0.27 in fertilizers, showing that equilibrium was maintained between the two nuclides. However, the radioactivity of Th series in phosphate rock has a high measurement uncertainty owing to being in a low concentration range. Therefore, it is difficult to judge the secular equilibrium in such cases.
2. Assessment of transfer characteristics and the radioactivity distribution in the production cycle of phosphate rock
Concerning the effect of radiation disequilibrium, the radioactivity of individual radionuclides was evaluated through ED-XRF for 238U and 232Th, and gamma spectrometry for 226Ra and 40K. In general, according to the geographical origin and chemical characteristics of the phosphate rock, the concentrations of 238U, 232Th, 226Ra and 40K are various. Table 1 shows the results for 23 types of phosphate rock distributed throughout Korea; the phosphate rock samples were imported from Nauru, Morocco, China, Togo, Egypt, Israel, Jordan, and Peru. The range of total radioactivity for each nuclide was as follows: 0.058–1.879 Bq·g−1 for 238U, 0.004–0.033 for 232Th, 0.051–1.720 for 226Ra, and 0.002–0.211 Bq·g−1 for 40K. The radioactivity of 238U and 226Ra in phosphate rock samples was much higher than that of 232Th and 40K. Among the 23 phosphate rock samples, eight of the samples showed that the radioactivity of 238U and 226Ra exceeded 1 Bq·g−1. These analytical results were within the range of the phosphate rock, which is produced and distributed all over the world [21].
To evaluate the transport characteristics and concentration distribution of 238U, 232Th, 226Ra, and 40K across the phosphate rock production cycle, various statistical approaches were applied in this study. The analysis of variance (ANOVA), Student-Newman-Kuels (SNK), Levene’s test, Welch statistic, and visualization using box-whisker plot were conducted using STATGRAPHICS Centurion (Ver. 17). The statistically compared results of the radioactivity at each stage are summarized in Table 2. For the clearance, the data that were less than MDA were converted into the observed minimum values of each nuclide, and the number of samples observed over the MDA are given in Table 2. A box-whisker plot was used to express and visualize the distribution characteristics of 238U, 232Th, 226Ra, and 40K (Figure 3). When the radioactivity of each sample group did not show homogeneity (p<0.005) in the Levene’s test, the Welch statistic as a robust analysis was also performed for a verification of the mean difference.
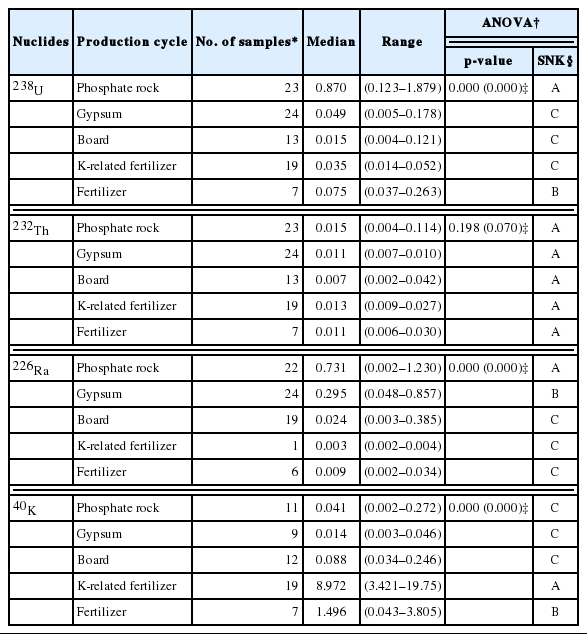
Statistical Summary of 238U, 232Th, 226Ra, and 40K (Bq·g−1) Activity in the Production Cycle of Phosphate Rock
In the production cycle of the phosphate rock, the radioactivity of 238U ranged from 0.123 to 1.879 Bq·g−1 with an average value of 0.870 Bq·g−1. 238U radioactivity in fertilizers, gypsum, k-related fertilizer and gypsum board ranged from 0.037 to 0.263 Bq·g−1, from 0.005 to 0.178 Bq·g−1, from 0.014 to 0.052 and from 0.004 to 0.121 Bq·g−1 with average values 0.0754 Bq·g−1, 0.049 Bq·g−1, 0.035 Bq·g−1, 0.015 Bq·g−1, respectively. As the results of the ANOVA in Table 2 show, the mean difference was statistically significant (p<0.005). The SNK results, which showed the rank of the mean of 238U in production cycles, revealed that the radioactivity in the phosphate rock and fertilizers is statistically higher than that in the gypsum, gypsum board, and k-related fertilizer. These results imply that most of 238U would be condensed in phosphoric acid through the chemical reaction between phosphate rock and sulfuric acid. On the contrary, since 226Ra has been easily enriched in gypsum, 226Ra radioactivity in gypsum is relatively higher (0.295 Bq·g−1 [range, 0.048–0.857 Bq·g−1]). However, as the results of the simple comparison for the concentration of 226Ra in this study show, 226Ra radioactivity in gypsum was about 2.5-times lower than that in phosphate rock. These results imply that the radioactivity in gypsum mixed with materials such as phosphoric acid and sulfuric acid was dramatically decreased owing to the dilution in manufacturing products. Moreover, the mean radioactivity of 226Ra in fertilizer and k-related fertilizer was statistically insignificant owing to the low concentration of 226Ra in these production cycles.
The median radioactivity of 232Th with respect to the production cycle is as follows: 0.015 Bq·g−1 (0.004–0114 Bq·g−1) in phosphate rock; 0.011 Bq·g−1 (0.007–0.010 Bq·g−1) in gypsum, 0.007 Bq·g−1 (0.002–0.042 Bq·g−1) in gypsum boards, 0.013 Bq·g−1 (0.009–0.027 Bq·g−1) in k-related fertilizer, and 0.013 Bq·g−1 (0.006–0.030 Bq·g−1) in fertilizers. The radioactivity levels of 232Th have been significantly not different (p=0.198). Because the radioactivity of 232Th are distributed in relatively low levels within the production cycle of phosphate rock, it was difficult to identify any particular transition pathways.
As shown in Figure 3, we determined that the median radioactivity of 40K was 8.972 Bq·g−1 in k-related fertilizer and 1.496 Bq·g−1 in fertilizers. However, because these products are compound fertilizers that may contain other sources of radiation, all of the radiation could not be accounted for by phosphoric acid. According to the Radionuclide and Radiation Protection Data Handbook [23], which is classified a total of five groups by the internal and external risks, the dose contribution of 40K is relatively small compared to the 238U and 232Th series. However, among the production cycle, the fertilizer contains a relatively high concentration of 40K. Therefore, the radiation effect of the fertilizer, as a by-product, is also not negligible.
Conclusions
In this study, through the ‘Act on protective action guidelines against radiation in the natural environment’, we investigated the characteristics of transport and radioactivity distribution of radionuclides in the production cycle of phosphate rock in Korea. The concentration of 238U is the highest in phosphate rock as a raw material, followed by fertilizer, phospho-gypsum, k-related fertilizer, and gypsum board, respectively. Thus, one may conclude that the metastatic pathway could be clearly identified. The concentrations of 238U and 226Ra in phosphate rock were very close to the registration standard (1 Bq·g−1). Therefore, systematic exposure control of a natural radioactivity exposure pathway of workers working with phosphate rock is needed. On the other hand, among the natural radionuclides, 40K shows a relatively a low contribution compared to the 238U and 232Th series at the total radiation dose. However, in fertilizer, the concentration of 40K is relatively very high. Therefore, the radiation effects for fertilizer manufacturing and use should also be considered as important management targets.
Notes
Nuclear Safety and Security Commission No. 10908 (2012.7.26.), Republic of Korea.